Sulfur is a major contaminant in the oil and gas industry that exists in various forms and requires separation from both water and process streams. In recent years the removal of sulfur species has received increasing attention because of safety concerns, the need to reduce emissions of sulfur oxides (SOx), and the fact that sulfur harms the combustion properties of fuels. The “sulfur recovery trail” starts with hydrogen sulfide (H2S) and mercaptans either present in raw hydrocarbon streams or arising in the petroleum refinery as a result of cracking or hydrogenation processes. The trail continues as H2S and mercaptans are captured by methods such as amine treatment, caustic scavenging, or sour water stripping, and ends with the conversion of H2S to elemental sulfur, sulfuric acid, salts or other materials.
In the many plants that make up the sulfur recovery trail, filtration and other separation processes are important in minimizing process upsets caused by the presence of contaminants. In fact, contamination control through separation is a key step in helping to maintain process control. Separation processes are often the first — sometimes the only — line of defense for avoiding many process variations and out-of-control episodes, and separation devices play critical roles in minimizing downtime and increasing systems reliability.
Studies at a number of plants worldwide reveal that many possess less than adequate separation and filtration systems, resulting in significantly higher operating costs. Many of the lessons learned along the sulfur recovery trail about the need for good separation are equally applicable to other parts of the chemical process industries (CPI).
Sulfur Recovery Trail
Sulfur in hydrocarbons has two major sources. It can be found naturally as covalent sulfides in hydrocarbon macrostructures, and it also occurs in other chemical forms in crude oil, connate water, produced water, natural gas and gas condensates. New sulfur species can also be generated when crude oil is processed. For instance, when water containing high levels of sulfate is used to aid crude oil production, sulfur reducing bacteria can turn sulfates into H2S. In some cases, H2S is produced by bacterial degradation of organic matter.
Removal of H2S is accomplished without much difficulty by amine systems, solvent systems, redox (reduction-oxidation) reactions, precipitation and chemical scavenging. Mercaptans (also called thiols or, in chemical shorthand, RSH), are somewhat closely related to H2S and are removed by fairly similar technologies. However, sulfur species that are covalently linked to organic systems are not as reactive as H2S and need to be transformed to H2S before they can be removed.
One of the most common ways to do this is through reduction with excess hydrogen, using catalysts, heat and pressure to drive the reaction to near completion. This is the basis of catalytic hydrogenation (also called catalytic hydrodesulfurization), in which sulfur residues (and to some extent nitrogen residues) in hydrocarbons are replaced by hydrogen. The sulfur combines with excess hydrogen to produce H2S, while nitrogen yields ammonia.
The resulting H2S-laden hydrocarbons are purified alongside hydrogenated hydrocarbons by amine units and other methods, followed, if necessary, by further processing to reduce mercaptan levels. H2S in water streams can be removed using chemical methods such as resin beds or additives, or by volatilization in a stripper (oftentimes with co-separation of NH3). In both cases the resulting gaseous H2S is generally sent to a sulfur recovery unit. At the downstream end of the sulfur recovery unit, remaining trace sulfur species can be further removed using a SCOT-type unit or other tail-gas recovery technologies before venting to the atmosphere. Taken together, these processes form the basis of the sulfur recovery trail (Figure 1), in which sulfur is generated, captured and converted for further use, transport, storage or disposal.
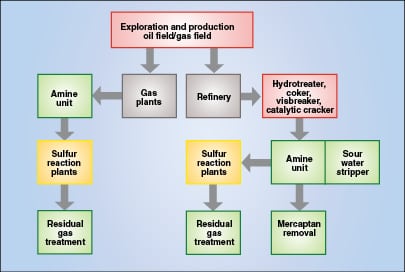
Role of separation processes
The sulfur recovery trail is a complex interlocked array of different units. Optimal performance of the trail is dependent on each of the units operating at its highest efficiency. Among the most basic needs for any process, system and plant in the sulfur recovery trail is the control of contaminants. Contamination control is essential in that it allows effective process control and enables units to operate consistently at an optimized cost and while maintaining or enhancing throughput.
Separation systems play a dual role in the process. They not only remove the daily contamination that exists in a normal process, but also protect the process from upstream upsets. The most common separation technologies in the sulfur recovery trail include demisters, filters to remove suspended solids from liquids, adsorption (activated carbon) beds, gas coalescers, flash tanks and two-phase/three-phase separators. Other systems are also utilized, but are more specialized and less common.
To cover all separation systems in a single article would lead to a lengthy discussion, so for simplicity we will look at one specific unit — the amine unit — which encompasses most, if not all, of the basic separations utilized in the sulfur recovery trail. The amine unit arguably suffers the most from contamination instabilities that considerably affect the performance, stability and reliability of the whole sulfur recovery process, but the concepts discussed here apply for the most part to other units in the sulfur recovery trail, and can be extended to the rest of the CPI.
Even well-operated amine units have uncontrollable factors that cause process upsets. Some of these relate to contaminants entering the plant via the gas or liquid to be processed, which is generally liquefied petroleum gas (LPG, C3/C4). To better understand its ramifications, it is important to understand what kind of contamination might be present in the feed. In addition to the gaseous hydrocarbon containing the H2S, the amine unit must handle many other components generated in the upstream oil production and transmission stages or in the various upstream refinery units.
Upstream gas production yields produced water loaded with a variety of water-soluble contaminants, and compressor lubricants with surfactant properties. Additives used to enhance production include demulsifiers, hydrate inhibitors, biocides, methanol and many others, and with the recent rise of shale gas availability we might expect new contaminants in both the upstream gas and the produced water. Clearly, proper separation technology is critical.
On the refinery side, contamination can take the form of coke fines, heat-stable salt precursors, corrosion products, water, additives (such as corrosion inhibitors and anti-fouling agents), carbon fines and compressor oil. Most if not all of these have some kind of surfactant activity or other detrimental effect. Together, these contaminants can cause a multitude of plant problems, such as amine contactor foaming leading to lower capacities, amine losses and low efficiencies. Additionally, some components can also cause issues such as amine degradation and formation of heat-stable salts.
Effective separation is especially challenging since all these contaminants can be in three possible states: solid, liquid and gas. In the solid state it is generally found that iron clusters predominate, along with carbon and coke fines, and in some cases sand, salts and oxides. Most of the liquid contaminants are lubricants, carried-over hydrocarbon products, carried-over amine solvent, cleaning products and water. This water can also contain soluble impurities, such as chlorides, sulfates and acetates among others. In the gas phase the predominant contaminants are substances such as hydrogen cyanide, oxygen and carbonyl sulfide. Other contaminants that can cause major plant upsets include methanol and BTEX (benzene/toluene/ethylbenzene/xylene).
Equipment in Detail Shortcomings of demisters
Inlet separation for gas streams is usually carried out using a knockout drum equipped with a demisting element, such as a mesh pad or vane pack, installed near or at the outlet of the vessel. These systems are typically horizontal in gas plants and vertical in petroleum refineries. The basic difference is that a horizontal drum offers increased residence time and holding volume, while a vertical drum has a smaller footprint. Some less-used technologies include conventional horizontal filter-separators as well as cyclonic separators.
However, conventional demisters are only adequate for removing large-diameter contaminant droplets. These separators were originally designed for bulk liquids removal (hence their alternative name of slug catchers). In addition, they are not designed for solids separation (usually done by a wet scrubber or a particle filter), with the exception of cyclonic systems that can remove large solid particles and some larger liquid droplets.
Most traditional approaches to separating liquid droplets, including demisters with mesh pads, vane packs or certain horizontal filter-separators, display rather low efficiencies when removing sub-micrometer aerosols from the gas streams. As a result, only a small number of plants have the necessary means to adequately condition sour gas entering amine plants.
The most challenging contaminants to separate in any gas stream are sub-micrometer liquid aerosols. These finely divided liquid droplets have diameters ranging from less than 0.1 µm to a few hundred micrometers. Droplets around the 0.1–1.0 µm range are the most difficult to remove due to the absence of a specific separation mechanism that yields high removal efficiency. Yet, as Figure 2 shows, about 50 wt.% of all liquid droplets in the gas stream are smaller than 1 µm, and 80 wt.% are smaller than 10 µm.
The lack of efficiency of most traditional separators relates to the aerosol droplet-size distribution, the flow configuration inside the separator, and the mechanism of liquid droplet interception. In other words, the separation medium is simply not capable of intercepting and coalescing sub-micrometer liquid droplets, followed by the liquid unloading required to maintain proper high-efficiency separation. As a result, most aerosol contaminants break out of the system almost intact, or are re-entrained in the gas flow.
Mesh pads suffer from flooding when excessive liquids are introduced and the mesh becomes saturated with liquid. This leads to efficiency losses through carry-over. These devices are also prone to fouling by solid particles, further reducing efficiency and causing considerable maintenance costs and pad failures. Movement of the mesh pad inside the vessel is somewhat common due to the difficulty of properly anchoring these devices to the vessel interior.
Vane packs offer better mechanical performance and lower differential pressure, but inferior separation efficiencies. Sub-micrometer liquid droplets in particular do not have enough momentum to properly contact the vane surface, so most small droplets are just carried with the stream.
In many vane packs and some mesh pads, one cause of inefficiency is the formation of interfacial layers. Vendors have tried to mitigate this through designs including double and single pockets, and also by combining vane packs with mesh pads. In general, however, none of these results in removal efficiencies adequate to protect sensitive equipment and processes.
Even when the separation medium is appropriate to the job at hand, the wrong vessel configuration can reduce separator performance. Gas routing inside the separator can be a source of significant inefficiency, and poor vessel design can actually shatter liquid aerosols into smaller sizes, adding more difficulty to an already challenging separation process. If the gas flow creates sufficiently large shear forces, large droplets will break up into successively smaller droplets until the distribution is stabilized by the balance of energy distribution, gravitational settling and shear.
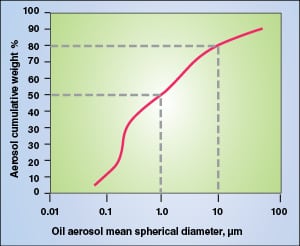
Microfibers perform better
Demisters are often used in sulfur recovery units, where care has to be taken to properly design and size them because these factors directly impact contamination removal and plant performance. So if mesh pads, vane packs, and cyclones should only be considered for liquid aerosols with droplet sizes well above 10 µm, what is the answer for sub-micrometer aerosols? Today, the technology of choice is built around specially formulated microfiber media. Properly designed and installed, a coalescer separator incorporating microfibers should remove 99.98+ wt.% of all droplets larger than 0.1 µm — at least in laboratory tests.
Such a high-efficiency sub-micrometer coalescer is a specialized piece of equipment that needs to be carefully designed depending on the flow, pressure, temperature, gas composition and contaminants. Many fabricators say that their systems are capable of removing sub-micrometer liquid aerosols, but most do not correlate these claims with actual performance. Only a small number of companies possess the proper technology to supply sub-micrometer gas-liquid coalescers.
The sub-micrometer coalescer should be installed as closely as possible to the unit or process it is intended to protect. It should be protected in turn by an upstream separator designed to remove large droplets — typically a conventional mesh pad. This extends the on-line life of the coalescer and minimizes operational costs, as a mesh pad is much less expensive to replace than a coalescer element.
A typical amine inlet-gas separator therefore has two stages mounted one above the other. Gas flows upwards into the lower stage, where large droplets are removed by a mesh pad, or sometimes a vane pack or cyclone. The gas then flows upward to the second vessel, across the microfiber coalescing medium, and the purified gas exits from the top of the vessel. Each stage has a liquid removal system comprising a level control and drain valves.
Typical campaign times for gas coalescing elements vary from a few months to two years, depending on the amount of solids entering the coalescing stage and the nature of the additives present.
Flash tanks and separators
Separation technologies based on pressure decay, velocity changes and residence time are among the most common separation systems used in oil and gas operations. All have the common theme of using simple concepts to attempt to solve a separation problem. One such example is the amine flash tank. This device removes off-gases by reducing the pressure of the rich amine solution downstream of an amine contactor. If designed correctly, the flash tank also provides limited liquid-liquid separation capabilities for hydrocarbon removal in the event that the stream has a high hydrocarbon content. However, removal will only apply to free hydrocarbons that separate from the amine solution over a timescale of minutes. Emulsified or dissolved contaminants are unaffected.
A number of different flash tank designs are available, but most feature poor design and short residence times. Some designs even incorporate metal mesh internals to promote coalescence and decrease residence time. Another reason why these apparently promising devices often provide poor to marginal results is their designers’ poor understanding of highly fouling, rich amine streams. Detailed design of the internals with respect to hydrocarbon separation is also an area of weakness: many vessel fabricators do not consider proper location and sizing for the internal box or weir that serves to remove hydrocarbons.
Two- and three-phase separators are similar to flash tanks with the difference that they are often larger in size and in some cases do not have any mist elimination devices at the gas outlet.
Many two- and three-phase separators are sized based on the correct parameters, but with a lack of understanding of liquid and solid loading. To use coalescing mesh pads correctly, these should be designed not only according to the gas velocity across the pad using the modified Souders-Brown equation, but also considering liquid (that is, water versus liquid sulfur) and solid (iron sulfide gels versus coke fines) properties and internal flow geometry. Any disregard of these aspects will invariably lead to element flooding and liquid carry-over or fouling with a differential pressure increase.
Liquid-liquid coalescers
Less commonly used, but quite powerful as separation devices, are liquid-liquid coalescers. These are often used to remove water or hydrocarbons from process streams. In the sulfur recovery trail they typically are installed in rich amine streams, sour water feeds, mercaptan removal unit outlets and many others. In sour water strippers and rich amine streams, hydrocarbon removal is important for plant reliability and is critical in enabling acid gases to be processed in sulfur recovery units (by minimizing hydrocarbons in the gas stream).
Liquid-liquid coalescers fall basically into two categories:
• Low-efficiency systems with metal internals
• Systems with microfiber internals
Both perform rather differently and should be used for different objectives. While coalescers with metal internals are good for separating free liquids and macroemulsions (about 100 µm and larger), coalescers with microfibers are more in line to separate microemulsions (100 µm and smaller), almost down to solubility limits.
Most liquid-liquid coalescers should be protected by particle filters or other solids pre-separation devices. These not only protect the coalescing pads or microfiber elements from solids plugging, but also disrupt solids-stabilized emulsions.
Suspended solids filtration
Most of the filtration in the sulfur recovery trail occurs in the amine unit (the sour water stripper, due to its highly corrosive environment, is another unit where filtration is important). The lean-amine filtration system is better defined as lean-amine conditioning, because filtration is not the only event taking place. Lean-amine filtration generally comprises three separate components, each with distinct functions and requirements. None of these can be replaced, eliminated or by-passed, and all of them are necessary for proper lean-amine conditioning. Rich-amine filtration is the most cost-efficient way to purify the amine solution. Its objective is to protect the amine solution itself, safeguard the lean/rich heat exchanger from deposition, reduce instability in amine regeneration, decrease corrosion rates in the regeneration stage, and reduce the solids burden on the lean-amine filters. It also provides a way to control high iron concentrations, which can cause unexpected foaming in the absorber through sudden sulfide formation (soluble iron entering the absorber with the lean amine rapidly generates insoluble iron sulfide upon exposure to H2S).
Filtration can be applied to either the full rich-amine flow or to a side stream. If structured packing is used in amine regenerators or absorbers, full upstream filtration is the best way to protect the packing and minimize solids deposition. Column packings make great particle filters.
Most plants find that rich-amine filtration brings significant process improvement and enhanced reliability. Prior to embarking on rich-amine filtration, however, a cost/benefit analysis and return-on-investment study, taking into account the costs of maintenance and filter disposal, are recommended. For amine solution filtration, the best technology is still disposable cartridges. These are relatively low in cost, easy to use, reliable, and ensure that contaminants are actually removed from the refinery. Other systems, such as automatic filters, generate significant secondary impacts in the form of the solids-laden backwash stream, which is generally sent to slop tanks or cokers. Automatic filters do not require frequent maintenance, but their filtration efficiency is low and in some applications their overall operating cost can be higher than that of cartridge filters. Automatic filters or backwash systems should preferably not be used in streams with a high fouling tendency, such amine units and others with complex mixtures of adherent suspended solids and hydrocarbons.
Centrifuges are one of the most promising emerging technologies for amine purification. However, drawbacks include high capital costs and maintenance costs, limited fluid capacity, poor efficiency on small particles, and challenges in operating under the highly fouling conditions that characterize amine streams.
Adsorption beds
Adsorption materials used to remove soluble contaminants include molecular sieves, alumina, salts, activated carbon, sand and fruit shells. Contaminants removed include certain heat-stable salts (“residues”) and their precursors, amine degradation products, certain dissolved hydrocarbons and foam-promoting species.
Activated carbon is perhaps the most common adsorbent. Many different types of activated carbon are available, with varying adsorptive powers for contaminant molecules of different types and sizes. Differences in the performance of different grades of activated carbon originate from the source of the material — coconut shells, wood, bitumen, fruit skins and many others — and the different activation processes used to enhance surface area and eliminate impurities from the pores. Some activated carbons also contain additives to enhance their mechanical strength.
Activated carbon for liquid streams is usually granular in form. Powder types are more suitable for some gas applications. Extruded carbons are now being used more widely; these tend to have slightly lower surface areas and somewhat higher differential pressures.
From a design perspective, activated carbon beds are simple, but require good understanding of their operation and failure modes. Fundamental aspects for efficient performance include correct carbon type, effective bed loading, proper liquid distribution to avoid channelling, adequate residence time, correct cross-sectional velocity, and a suitable ratio of bed diameter to length. Activated carbon beds are not filters (see box above): they are not designed to separate suspended solids, nor free or emulsified hydrocarbons. The presence of these types of contaminants will rapidly render the bed useless.
Activated carbon beds release carbon fines (small carbon particles) from fractured carbon granules, and it is necessary to capture these solids before they reach any downstream operation. For amine plants this is generally the absorber tower, where the presence of carbon particles will cause foaming, fouling, and depending on fluid velocity, erosion corrosion. Any of these can lead to low sweetening efficiency and amine losses. More often than not, carbon particles are also found in the rich amine stream, inside and downstream of the rich-amine flash tank, and even in the regeneration stage.
Amine recovery
Amine losses have become an area of great interest lately due to the considerable economic costs of amine replacement. Often overlooked is the fact that the lost amine will end up in a downstream process unit, wastewater treatment facility or slop oil tank. Any of these destinations will have a significant impact on equipment reliability, process stability and economics.
Methods of recovering lost amine include knockout drums with mesh pads or vane packs, water washes, coalescers and other more specialized technologies. Removal of amine from treated liquid-hydrocarbon streams is important due to the high emulsification rate of the amine in the hydrocarbon, in addition to its solubility and mechanical entrainment. In this case, water washes and other separation systems have been implemented with varying degrees of success. Conventional water washes tend to use large amounts of water and have low amine-recovery efficiencies. Today, there are newer and more technologically advanced systems called extractive separation technologies. These devices are capable of recovering amines much more efficiently and with lower costs, reduced water use and minimal footprint.
Typical Fluid Contamination Scenarios
In principle, a well-operated amine unit has no need for filtration. Such an ideal system is seldom encountered, however. In the real world, filtration and related separation technologies may be the only line of defense against serious upsets in amine units, and in other plants too. Deploying the best and most advanced contamination-control devices helps to ensure process stability, equipment reliability, and enhanced throughput. Separation technologies mitigate a series of problems commonly found in the sulfur recovery trail, such as the following:
Fouling. This is deposition of solids and hydrocarbons along with other components to form a coating over equipment surfaces typically in hot, low-velocity locations, such as heat exchangers and reactor columns. Fouling has many mechanisms, but there is some agreement that there are two main routes: (a) a free radical polymerization, condensation or decomposition of dissolved species present in the stream, or (b) deposition of suspended matter present in the stream. Today, fouling is generally prevented by chemical means (free radical inhibitors such as hindered phenols) or by mechanical means, such as filtration. Both methods can be effective, depending on the fouling mechanism and process conditions. Fouling also leads to energy losses and flow reductions and is a source of major maintenance efforts.
Corrosion. Typically, where there is fouling, the next natural progression is electrochemical corrosion beneath the fouling deposit. This is caused by elevated local concentration of corrosion initiators and the formation of electrochemical cells. Usually if fouling is minimized, corrosion rates will be lowered as well. Some plants also suffer from erosion corrosion, which occurs when hard, dense particles strike the material surface, removing passivation layers and the metal beneath. Corrosion mechanisms are often complex.
Heat-stable salts and amine decomposition. Heat-stable salts are amine salt contaminants that do not decompose under normal regeneration conditions. Despite the name, some “heat-stable” salts will break down at temperatures higher than those routinely found in the plant. It is believed that the formation of many of these salts is accelerated by the presence of suspended solid contaminants and mediated by dissolved metal ions such as iron. Solid surfaces possess many active sites for heterogeneous reactions and are also rich in metal species that can catalyze salt formation, which increases the concentration of heat-stable salts and promotes amine decomposition. In many cases, reducing suspended solids and hydrocarbons in the amine solution will decrease the rate of heat-stable salt formation.
Foaming. This is generally produced by the association of gases and liquids stabilized by surfactants, which lower surface tension at oil/water interfaces. These surfactants can be solids (such as micrometer-size iron sulfide particles) or individual molecules (such as compressor lubricant). Foaming invariably leads to amine loss and lower efficiency. Removal of solids and hydrocarbons greatly reduces foaming and hence the need for antifoam additives. In amine units, however, other factors can also lead to foaming, and these can have many root causes. Hence, proper plant evaluation (process, equipment, feed composition) is required to determine the source of foaming. Antifoam agents should be used with caution as in large doses they may actually promote foaming, and they may separate out in certain filtration systems. Remember that antifoam agents treat only the symptom, not the cause.
Regenerator protection and acid gas quality. Filtration and separation systems on the rich-amine stream are designed to protect the regeneration section of an amine plant and to protect the sulfur recovery unit by facilitating the delivery of good-quality acid gas. This is done by ensuring that the amine is free of contaminants that would foul the rich/lean heat exchanger, increasing the reboiler duty and generally causing corrosion at the bottom of the regenerator. More plants are now adopting rich-amine filtration in addition to lean-amine filtration. In fact, both stages are not only necessary but complement each other. Removal of contaminants, such as suspended solids and hydrocarbons, from the rich-amine stream ensures a better acid-gas quality with better, smoother and easier amine regeneration. The ingress of contaminants such as hydrocarbons into the sulfur recovery unit not only produces soot, but can also seriously compromise the catalyst’s physical integrity. Hydrocarbons compete with H2S in the initial oxidation stage, resulting in considerable variability and mismatched oxygen demand in the stoichiometry of the modified Claus reaction.
Low sweetening efficiency. Filtration is designed to remove suspended solids from the amine solution. It is known that lean amine with a high solids content is less able to transfer H2S efficiently. This is caused by multiple layers of solids at the interface of the gas (or liquid) and the amine solution, essentially blocking mass transfer. Good-quality amine solutions with minimal contaminants perform much better, promote process stability, prevent equipment damage, save energy, and so lower the overall annual operational cost of the plant.
The following sections present a compendium of recommendations and guidelines for proper filtration and separation in the sulfur recovery trail. This is not a definitive recipe, because every plant is different, but it is a fairly comprehensive summary of experience gained in many years of field tests around the world. Many of the messages here will have application beyond the sulfur recovery trail.
Contamination Sources in Sulfur Recovery
Hydrogenation plants. Inlet contamination, recycled hydrogen contamination and outlet contamination
Amine units.Inlet contamination, outlet carryover and amine solution contamination
Sour water plants. Inlet contamination, outlet contamination, and acid gas contamination
Sulfur reaction plants (including sulfur recovery units). Inlet contamination
Mercaptan removal plants.Inlet contamination, outlet contamination and caustic solution contamination
Filtration and separation systems considerations
• Knowing the end goal, and the constraints on plant operation and cost
• Protecting equipment from fouling or degradation
• Meeting fluid specifications
• Enhancing equipment reliability and stabilizing the process
• Ensuring environmental compliance, reducing waste and emissions
• Lowering maintenance effort and cost
• Reducing overall contamination removal costs
• Enabling the unit to increase throughput
Criteria for selecting filtration and separation systems
• Analyze the process stream for contaminant types and concentrations
• Understand particle sizes and shapes
• Sample over a period of time to detect process variations
• Understand the contamination sources; this may lead to different solutions
• Filter efficiency ratings form a reference point from which to start — perform adjustments with the unit operational and with on-line sampling
• Consider future expansions
• Carefully choose the location for installing the system
• Consider maintenance and environmental aspects
Filtration and separation systems failure modes
• Improper technology for the application
• Incorrect compatibility (chemical, thermal or mechanical)
• Deficient vessel design
• Inappropriate or deficient sealing surfaces
• Incorrect media and efficiency
• Lack of or incorrect maintenance
• Instrumentation deficiencies (or lack of necessary instrumentation)
• Change in feed conditions
Concluding remarks
Many years of field experience teach that a key step in process control is proper control of contamination. Most plants that do not take this step struggle with high operational costs and low reliability, in addition to many detrimental technical, economic and environmental aspects.
There is no significant disadvantage to implementing enhanced separation and filtration besides a marginal increase in capital cost. One might tend to believe that cost will be prohibitive, but experience shows that this is not the case, and operational costs can actually be lower. On the other hand, there are real and serious issues involved with neglecting separation and filtration systems, using systems with deficient designs, or simply not giving the proper attention to contamination control. Invariably, any capital savings from low-cost separation and filtration will ultimately lead to exponentially higher processing costs, low reliability and frequent unit upsets.
It is also important to understand that each plant and process has its own equilibrium point where the cost of contamination control is acceptable and the residual contamination level is tolerable. Users, engineering firms and suppliers have the responsibility to be involved in finding such balance, with the objective of supplying the right separation and filtration solution for each individual plant.
A holistic understanding of the case, and why a given separation system is required, is critical in designing, troubleshooting, optimizing, operating and maintaining systems. This will provide positive process and economic benefits, allowing each plant to take full advantage of the installed process capabilities and to maximize throughput.
Author

David Engel is the senior consultant and co-founder of Sulphur Experts, Filtration Division (www.sulphurexperts.com) and managing director of Nexo Solutions LLC (21 Waterway, Suite 300, The Woodlands, Tex. 77380; Phone: 832-296-6624; Email: [email protected], Website: www. nexosolutions.com). He has more than 20 years of industrial experience in many areas ranging from sensors, corrosion-resistant materials to separation technologies. He is the inventor in more than 15 U.S. patents, and has developed new businesses and technologies, and executed projects, for Eastman Kodak, Eli Lilly, Pentair, General Electric and Sulphur Experts. He holds a B.S. in industrial chemistry and a Ph.D. in organic chemistry, and is Six-Sigma certified. He is a member of the American Chemical Society (ACS), and the Gas Processors Association (GPA). He currently is the president of the American Filtration and Separations Society (AFSS), Southwest Region.