Hydrogen is poised to be a cornerstone of the global energy transition and its production via electrolysis has ignited demand for high-performance electrolyzer units
Technological and economic advances have brought hydrogen to the forefront of sustainability strategies in many industries, with end users hoping to capitalize on the promise of significantly reducing, or altogether eliminating, CO2 emissions.
Globally, many organizations are developing sustainability and energy initiatives centered around hydrogen, including projects in the U.S., Canada, Saudi Arabia, Denmark, Austria, New Zealand, Australia, Singapore, Germany, Chile, Spain, China, Portugal and Japan.
Electrolyzer basics
Much of the activity surrounding hydrogen today involves electrolyzers, which are modular processing units wherein electrical current is applied to split water molecules into hydrogen and oxygen. When powered by renewable electricity sources, such as wind or solar power, electrolyzers produce emissions-free, or “green” H2.
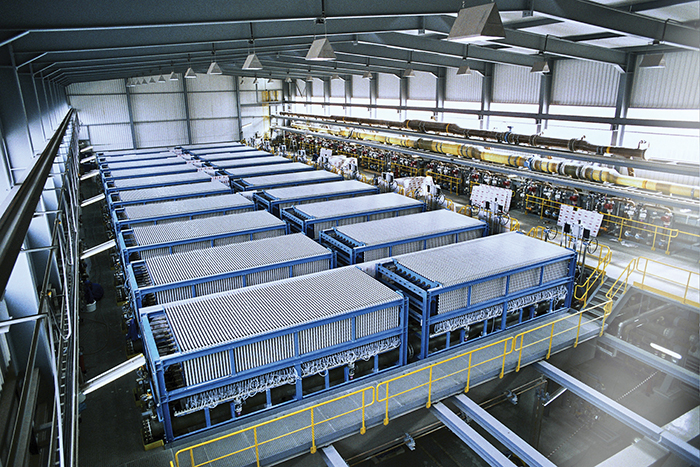
FIGURE 1. Electrolyzer manufacturers are expanding capacity to satisfy growing global demand
In recent years, production of electrolyzers has ramped up significantly to meet global demand for green H2. In June, thyssenkrupp Industrial Solutions AG (Essen, Germany; www.thyssenkrupp-industrial-solutions.com), in partnership with De Nora S.p.A. (Milan, Italy; www.denora.com), expanded its manufacturing capacity for electrolyzer units (Figure 1). “We now have the capability to build electrolysis plants with an annual capacity of 1 gigawatt, and we will expand our capacities even further,” explains Christoph Noeres, head of energy storage and hydrogen at thyssenkrupp. These electrolyzers are offered as prefabricated skid-mounted modules (Figure 2), which can be combined to easily scale up production capacity. Scaling up the electrolyzer capacities, says Noeres, will help to realize economically promising value chains, not just for the large-scale production of green H2, but also for the subsequent manufacture of sustainable chemicals, such as ammonia and methanol. “Green H2 will play a central role in achieving greenhouse-gas neutrality, as well as the establishment of a closed-loop economy,” adds Noeres. On the horizon, thyssenkrupp is focusing its development projects on regions with favorable conditions for power-to-x applications. Earlier this year, the company announced that its electrolysis plants will be able to link to the German electricity market via E.ON’s virtual power plant, effectively acting as large-scale buffers to stabilize the power grid. For this ambitious milestone, the electrolyzers had to meet several load-change qualification criteria detailed in the grid codes of transmission operators, demonstrating that they exhibit sufficient response speed and flexibility to participate in the energy-balancing market.
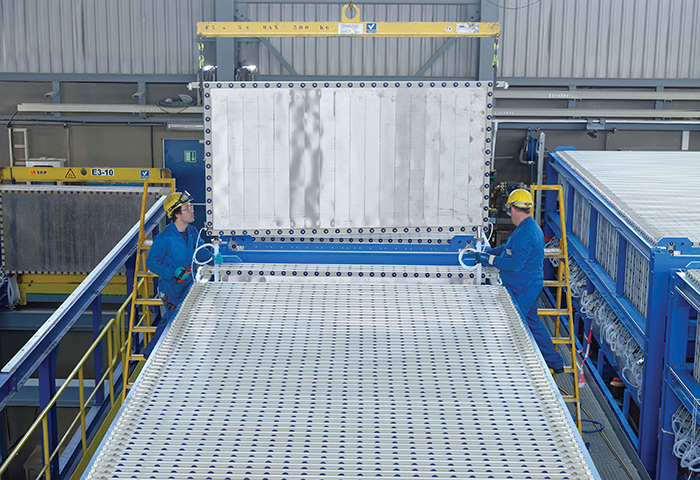
FIGURE 2. Their modular nature makes electrolyzers suitable for installations both large and small
There are two primary types of electrolyzers on the market — alkaline and proton-exchange membrane (PEM). Other emerging electrolysis technologies, which are still primarily in the development phase, include anion exchange membrane (AEM), solid-oxide electrolyzer cell (SOEC), protonic ceramic electrochemical cell (PCEC) and photoelectrochemical (PEC) water splitting. In an alkaline electrolyzer, the water is split into its constituents in the presence of a caustic electrolyte solution — frequently potassium hydroxide (KOH). The water-splitting reaction in a PEM electrolyzer gleans its electrolytes from a catalyst that is applied to a polymeric membrane.
Alkaline electrolysis is the more established technology, and alkaline electrolyzers typically are more affordable, but PEM electrolyzers bring some added value via a more rapid response to changes in power. Furthermore, PEMs are often seen as a safer option, since the membrane provides a physical barrier between the produced H2 and O2.
Lower costs, higher performance
Although electrolyzers are not new, recent development work and industry trends have made them much more attractive when compared to conventional H2 production from natural gas via steam-methane reforming (SMR), says David Bow, senior vice president, corporate business development & strategy, Nel Hydrogen (Wallingford, Conn.; www.nelhydrogen.com). “The electrolyzer industry has dropped its capital costs by as much as 75% in the past 2 to 3 years, which has been driven mainly by market need for larger systems and innovation in system design and manufacturing,” explains Bow. The proliferation of low-cost renewable energy is also an enormous driving force, alongside pressure to meet corporate and government sustainability targets. “An SMR will produce 10 to 12 tons of CO2 per ton of H2 produced. Now, low-cost renewable electricity can be supplied to make green H2 with zero CO2 emissions,” says Bow. A major goal for electrolyzer providers is to achieve “fossil parity” — meaning the electroyzer can produce green H2 for the same price as using an SMR with natural gas (“gray” hydrogen).
After achieving considerable cost reductions, Nel is now focusing more of its developmental efforts into improving electrolyzer efficiency and performance, including work to decrease the amount of precious metals (like platinum and iridium) in PEM catalysts, and advancements in electrode technology for alkaline systems.
Geography is an important factor when comparing the economy of SMRs with electrolyzers. In some areas, where natural gas feedstock for SMR units is scarce, natural gas needs to be shipped; or H2 transported via tube trailers or in liquid form in tank trucks, which is very inefficient and CO2 -intensive. “Since H2 is such a light molecule, a full tube trailer truck can carry only around 350 kg. Furthermore, there are considerable losses when storing hydrogen as a liquid, since it vents off as temperatures change,” explains Bow. This makes onsite generation of H2 a much more attractive proposition for major hydrogen consumers, such as ammonia plants, methanol plants and petroleum refineries.
While SMRs are by far the dominant technology, many chemical processing sites are turning to electrolyzers to help augment SMR capacity and increase plant flexibility, since electrolyzers can operate efficiently over a large turndown ratio and are readily scalable. Bow mentions an example of a large chemical manufacturer who was purchasing H2 from a nearby SMR unit and found that their demand had outgrown the SMR’s capacity. “They looked at buying another SMR unit versus transitioning to electrolyzers or transporting liquid H2 in tanker trucks and found that electrolyzers in series provide more efficiency at a lower cost,” says Bow.
Nel has undertaken a variety of pilot-scale tests for different H2 applications, helping sites to transition from gray to green H2. “Many, if not all, major ammonia producers are looking at some level of electrolysis testing. We have a wind-to-ammonia project in Minnesota that has been running for several years and many more in the pipeline. We’ve also sold a number of large-scale alkaline electrolyzers for production of ethylene and sugar alcohols, both of which consume large amounts of H2 in the process,” says Bow.
Nel Hydrogen is one of several recipients of funding under the H2@Scale initiative funded by the U.S. Department of Energy (DOE; Washington, D.C.; www.doe.gov), through the Office of Energy Efficiency and Renewable Energy’s (EERE) Hydrogen and Fuel Cell Technologies Office (HFTO). In July, $64 million in funding was awarded to 18 projects supporting H2@Scale’s goals of advancing the U.S. hydrogen economy. Nearly $15 million of the most recent round of funding went to projects focused specifically on manufacturing electrolyzers. “An advantage of electrolyzers is their suitability with intermittent renewable-power sources, such as wind and solar. Instead of curtailing power, electrolyzers can be used to make H2 for either energy storage or for other value-added end-use applications, such as manufacturing chemicals or steel,” explains HFTO director Sunita Satyapal.
For electrolyzers, H2@Scale’s main goals involve improving efficiency and durability while reducing overall costs. As electrolyzer technologies have progressed, Satyapal points out a trend toward more holistic and collaborative development projects. “Instead of looking at specific components, like catalysts or membranes, much of the current work deals with the integration of materials and manufacturing processes, and how we might be able to integrate them to be manufacturable at scale,” she says. “An example of a unique area we have been funding is quality-control methods. Ideally, if we are going to ramp up electrolyzers to gigawatt scale, the components wouldn’t be manufactured in batch processes, so we are looking at higher-throughput continuous processes, such as roll-to-roll, as well as high-speed inspection over large-area components to find defects that could impact durability.” Some of the other major areas of development include: membrane-coating techniques and simplifying membrane fabrication; optimizing the porous transport layer; and reducing precious-metals content. In addition, H2@Scale is working on two first-of-their-kind nuclear-to-H2 projects in the U.S.
H2@Scale is focusing on multiple hydrogen production, storage, distribution and utilization needs, including PEM electrolyzers, which are gaining traction in the market, but still have potential for major cost reductions. Feedwater quality is another emerging area of research for the project, says Satyapal. “We have a few early-stage projects investigating the ability to use dirty water or salt water, as opposed to requiring high-purity water for electrolysis,” she adds. “We also have a first-of-its-kind project in the U.S. where we are producing H2 with an electrolyzer and using a biological system to make renewable methane with H2 and CO2,” says Satyapal. Other application areas for the H2@Scale project include datacenter power, maritime transport, steelmaking, ammonia production and heavy-duty trucks that can help ramp up scale and drive down the costs of hydrogen production and infrastructure.
Synergy with natural gas is another area with significant interest, specifically in blending H2 and natural gas, with the potential to inject hydrogen into natural-gas pipelines. However, with H2 blending, materials compatibility can be a major concern depending on materials used, and much research activity involves the effects of hydrogen on embrittlement and its impact on both metals and polymers, as addressed by DOE’s H-Mat consortium.
A significant milestone in H2 blending took place in July, when Baker Hughes (Houston; www.bakerhughes.com) and Snam (San Donato Milanese, Italy; www.snam.it) completed testing of the world’s first “hybrid” hydrogen turbine designed for a gas network, with the ultimate goal of injecting H2 blended with natural gas into Snam’s current transmission infrastructure.
Advancing PEM electrolysis
Hoeller Electrolyzer GmbH (Wismar, Germany; www.hoeller-electrolyzer.com) has developed an optimized cell-surface technology for compact PEM electrolyzers (Figure 3) that reduces the amount of precious metals required and increases operating pressure. Hoeller is designing its PEM cell stacks with demanding installations in mind, such as integrating the stack directly into the head of a wind turbine. “The key advantage of PEM electrolysis is that the H2 production almost instantaneously changes with the energy provided, so processes with a changing need for H2 are an ideal match,” says Matthias Kramer, chief financial officer at Hoeller. According to Hoeller, its stacks can handle load changes from 0 to 100% of nominal load within seconds. While PEM is versatile in the face of shifting demand, Kramer also emphasizes its ability to operate continuously. Furthermore, the stack is capable of pressurization to 50 bars or higher, making direct storage more convenient. Hoeller’s proprietary PEM technology was demonstrated in a proof-of-concept at the Fraunhofer Institute for Solar Energy Systems (ISE; Freiburg im Breisgau, Germany; www.ise.fraunhofer.de), and Kramer says that the company expects to install a prototype unit by the end of 2020. Discussions are also in place regarding a pilot project for the new PEM stacks at a wind farm in Schleswig-Holstein, Germany.
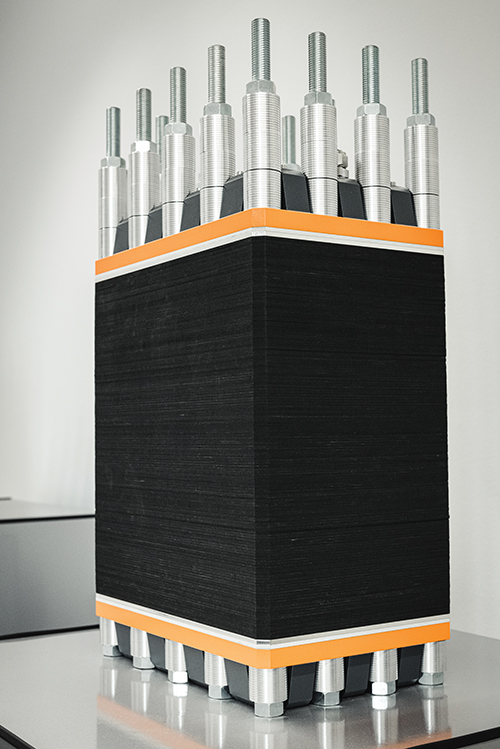
FIGURE 3. These compact electrolyzer units are designed for installation in challenging locations, such as the head of a wind turbine, for streamlined
energy storage
AEM electrolysis
One emerging technology for H2 production is anion-exchange membrane (AEM) electrolysis (Figure 4). AEM is somewhat of a hybrid solution combining the benefits of PEM and traditional diaphragm-based alkaline electrolysis, explains Oliver Conradi, who specializes in membrane research at Evonik Industries AG (Essen, Germany; www.evonik.com). “Alkaline electrolysis obviously involves very basic conditions, while PEM involves an acidic environment. These respective pH values require certain materials. In alkaline conditions, you can use cheaper materials, such as stainless steel and nickel, whereas with PEM, you must use platinum or other precious metals for the catalyst, and the electrochemical cell must be based on titanium, so the investment cost for PEM is much higher,” explains Conradi. However, PEM systems overcome some of the fundamental limitations of traditional alkaline electrolysis — due to the specific cell design in alkaline systems, current density and efficiency are limited, and it is more difficult to pressurize an alkaline system, meaning that an additional compression step is typically required. “In PEM units, the dense membrane makes it easier to pressurize the whole system. With AEM, you can fundamentally combine the advantages of both state-of-the-art technologies, while you compensate for their drawbacks,” says Conradi, noting that the primary hurdle in developing an effective AEM system is developing a suitable polymeric membrane material that can withstand alkaline conditions.
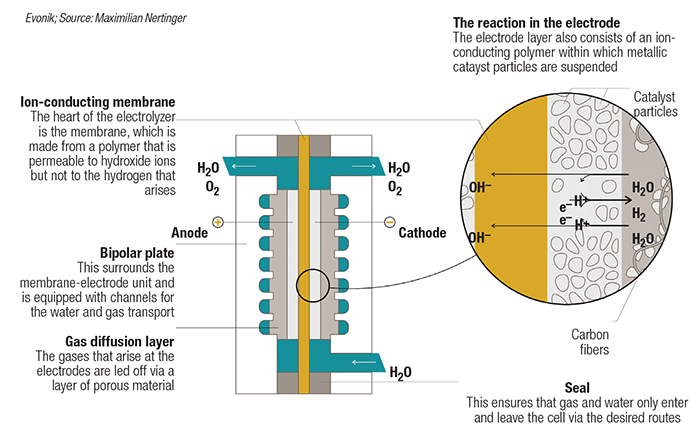
FIGURE 4. AEM electrolyzers are poised to combine the benefits of alkaline and PEM electrolyzers, which are currently the dominant technologies on the market
A particular area of focus is on the cationic moiety, which is responsible for transporting the hydroxide ions from the cathode to the anode. In addition to stability in an alkaline environment, the polymer must also provide high ion conductivity and stability under pressurized electrolyzer conditions. Inspired by an existing membrane technology for gas separation, Evonik has developed a new polymer chemistry featuring a proprietary ion-conducting cationic moiety. As part of the AEM-focused Channel consortium, Evonik is expanding production of the polymer and also scaling up membrane fabrication on a pilot coating line. “The consortium is building an AEM electrolyzer to demonstrate that the membrane and other components work under challenging conditions,” explains Conradi. The group’s first AEM demonstrator unit is at the laboratory scale, where test protocols are being run to reflect real-world conditions. “The next milestones will be proving system reliability and scaling up the stack sizes, while also scaling up membrane processing,” he continues.
Downstream H2 processing
Although electrolyzers have made strides in efficiency and cost, the produced H2 still often requires post-processing steps, such as compression, dehydration or purification. “Electrolysis stacks usually do not produce hydrogen that is directly suitable for use. If you want to store, distribute or utilize the produced hydrogen, contaminants need to be removed,” says Jordi Zonneveld, manager of the hydrogen portfolio at Frames Group (Alphen aan den Rijn, the Netherlands; www.frames-group.com). “Since PEM technology uses only ultra-pure water, the only contaminant is water, and potentially a very small amount of oxygen. Alkaline electrolysis uses a KOH solution as the process fluid, and therefore traces of KOH in the produced hydrogen need to be removed, as well.”
Depending on the gas flow and purity requirements, there are several steps that may be required to prepare H2 for its end-use applications. For instance, says Zonneveld, knock-out drums with demisting internals and optional gas-cooling equipment are generally used as a first step to bring hydrogen purity up to 99.9%. Then, if higher purity is required, a molecular-sieve unit (Figure 5) may be required. He also mentions that dehydration using triethylene glycol — a common technology for natural-gas processing — has shown potential for H 2 purification, but there have not yet been any large-scale H2 applications.
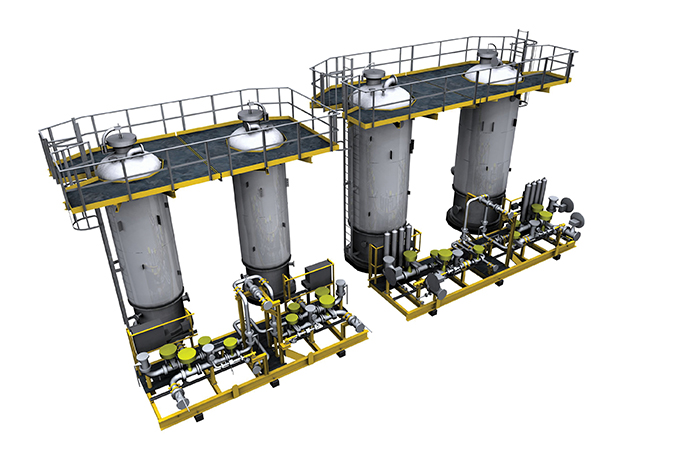
FIGURE 5. A molecular sieve unit may be installed downstream of an electrolyzer for dehydration purposes
Compression of H2 also introduces unique challenges. “H2 has a very high energy density per mass, but a very low density, so compressors are needed downstream of electrolyzers to compress the H2 for efficient storage and transportation,” says Stefanie Peters, managing partner at Neuman & Esser Group (NEA; Übach-Palenberg, Germany; www.neuman-esser.de). The low molecular weight of H2 also poses issues. “Turbomachinery faces significant problems in capturing the H2 in the compression chamber, and only positive-displacement machinery like piston and diaphragm compressors are suitable for efficient compression to required H2 discharge pressures,” add Peters. For instance, dry-running piston compressors can achieve discharge pressures up to 300 bar. When equipped with lubricated cylinders, the discharge pressures are potentially as high as 700 bar, but this option introduces trace amounts of oil contamination, so in cases where no contamination is acceptable, oil-free diaphragm compressors are the preferred high-pressure option, as they can achieve more than 5,000 bar discharge pressure.
As the demand for electrolyzers and green H2 continues to grow, technological improvements, not just in the electrolyzers themselves, but also in post-processing, will continue to be vital areas of research and development work.