Water scarcity is one of the most serious global challenges of our time. Desalination and water reuse are effective and reliable means to provide new water resources. Among the many methods available to treat water, reverse osmosis (RO) has widely demonstrated superior reliability and cost-effectiveness at removing dissolved species, such as salts and trace contaminants. This article reviews the development and state of the art of RO for industrial water treatment, wastewater treatment and drinking water production. It also reviews technological advances that have improved process performance, reliability and reduced cost, and describes currently available components and methods. Finally, it considers the prospects for future advances in the field.
Water scarcity and desalination
The world’s freshwater resources are under tremendous pressure. Over one-third of the world’s population lives in a water-stressed country, and by 2025, this figure is predicted to rise to nearly two-thirds of the global population [1]. Industrial operations consume an estimated 60% of all freshwater withdrawals in developed countries like the U.S. Together, industry and agriculture are responsible for about 90% of the freshwater consumed globally [2]. Efficient and sustainable water treatment methods for industrial and agricultural water supply are imperative.
Water should be conserved, recycled and reused to reduce the stress on water supplies, but this will not meet the increased demand posed by population and economic growth. The treatment of salty water resources and wastewater reuse offers new and reliable sources of freshwater, without impairing existing freshwater resources. Today, an estimated 300 million people in 150 countries already rely on desalinated water. In 2016, the global water production by desalination is projected to exceed 10 trillion gal/yr (38 billion m 3/yr) — twice the rate of global water production by desalination in 2008 [3].
Early desalination and water purification methods included distillation, which uses large amounts of energy to evaporate freshwater from saltwater [4]. Distillation plants for desalination still operate today in some regions of the world where energy is abundant and inexpensive. However, the vast majority of desalination and water-purification plants that have been constructed recently or are planned employ RO technology.
Thanks to technological improvements over the last 20 years, the cost to produce freshwater with RO has been reduced by a factor of four or more, and the process has become a reliable component of municipal and industrial infrastructure (Figure 1). At present, RO is the most energy-efficient and versatile technology for desalination and water purification and is the benchmark for comparison for any new water-purification technology [5,6].
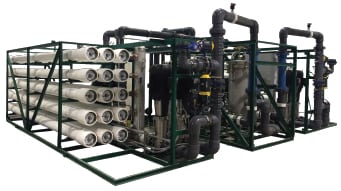
RO is most commonly known for its use in purifying drinking water from seawater, brackish water or contaminated water, where RO removes salt and other dissolved or suspended materials from feedwater. Many are surprised to learn that its use in other applications is widespread and well-established. For example, RO is used to remove minerals from boiler water at power plants, to clean effluent and brackish groundwater, and for concentrating food liquids, such as milk.
Reverse osmosis principles
Osmosis is the natural movement of water from an area of high water concentration (low salt concentration) through a salt barrier to an area of low water concentration (high salt concentration). Flow is driven by the difference in osmotic potential of the two solutions, quantified as osmotic pressure. Applying an external pressure to reverse the natural flow of water through the barrier is RO.
The process of osmosis through a semipermeable membrane was first observed in 1748 by Jean-Antoine Nollet. RO was known in the 1950s, but was not practically demonstrated until the early 1960s, with the discovery of asymmetric membranes at the University of California at Los Angeles (www.ucla.edu) by Sidney Loeb and Srinivasa Sourirajan [7]. These membranes were characterized by a thin “skin” layer supported atop a highly porous and much thicker substrate. This basic structure remains the basis of modern RO membranes.
When a salt solution is pressurized against an RO membrane, impurities are retained on the pressurized side of the membrane as brine and purified water flows through the membranes as permeate. RO requires flow across the membranes, known as crossflow, to keep the membrane surfaces clear of concentrate and allow continuous and almost constant flow of permeate. This differs from conventional filtration processes, in which impurities embed in the filter or build up as a cake that must be backflushed or removed periodically to restore productivity.
The minimum pressure required to separate pure water from impure water can be considered as a barrier. The height of this barrier depends upon the osmotic pressure or osmotic potential of the water, which in turn depends upon its salinity and composition. Saltier water has a higher osmotic potential and requires more pressure to desalinate. To drive permeate through membranes at reasonable fluxes, the osmotic potential must not only be met, but overcome. This overpressure depends partly upon the permeability of the membranes. In addition, the salt concentration immediately adjacent to the membrane surface is elevated, which increases the osmotic barrier above that in the bulk of the saltwater — a phenomenon known as concentration polarization. Concentration polarization is reduced with crossflow.
Other non-idealities in RO systems that elevate energy requirements include resistance to flow through the membranes, mechanical and volumetric efficiency losses in pumps and viscous friction losses in flowing water. Also, membrane surfaces can be blocked with contaminants, including organic and inorganic foulants or salts that precipitate out of solution.
For RO treatment of brackish, industrial and wastewater (collectively referred to here as industrial RO) salinity levels and the corresponding feed pressure requirements are typically lower than those for seawater RO. Therefore, energy requirements are usually not a major cost consideration. However, the cost and practicality of brine disposal and the need for the process to handle feed composition changes are more significant in industrial RO applications. To limit brine production and achieve a high yield of purified water, industrial RO systems operate at high recovery rates, where recovery is defined as the ratio of permeate flow to source-water flow. However, high recovery increases the risk of membrane contamination, presents challenges for maintaining crossflow, reduces permeate quality and can reduce the flexibility of the process to handle feedwater variations.
Many of these challenges have been met with emerging technologies that enhance the utility of RO.
RO systems
RO membranes are incorporated into systems that deliver source water to the membranes, apply crossflow that sweeps concentrate from the membrane surface, and provide a pathway for conveying water that permeates through the membranes. The primary components of an RO system are the membrane elements, the pumps and the devices used to manage flow and pressure. Typically, a high-pressure pump provides both the driving force for the separation and the crossflow, but several current process designs use a circulation pump to drive crossflow, so that the role of the high-pressure pump is reduced to pressurizing the system and driving permeate flow.
RO process configurations
A typical system for industrial RO is shown in Figure 2. A high-pressure (HP) pump feeds the system. Pressure vessels containing multiple membrane elements are arrayed in parallel. Each pressure vessel contains six or seven membrane elements in series, with the concentrate of each element being fed to the subsequent element. One stage typically achieves 50% recovery, and higher recovery rates require multiple stages. The productivity of multiple stages are balanced by throttling the permeate flow from front stages and boosting feed pressure to later stages with a booster pump (BP). The number of pressure vessels arrayed in parallel in each subsequent stage is reduced to maintain sufficient crossflow in later stages. Finally, brine is throttled out of the system through a valve or a device that regulates system pressure.
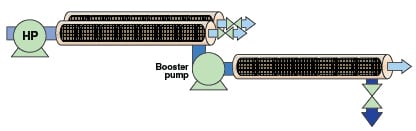
At 50% recovery per stage, four stages are required to achieve over 90% total recovery. Multiple stages add design and operational complexity and reduce process flexibility. Alternately, recovery can be increased by continuously returning some of the brine to the membrane feed. However, the increase in feed, brine and permeate salinity caused by brine recirculation usually undermines the benefits it provides.
Emerging closed-circuit (semi-batch) RO processes, which utilize brine recirculation in a batch-like operation, provide a new means to achieve high recovery without multiple stages or reduced permeate quality [8,9]. Such a process is illustrated in Figure 3. A high-pressure pump feeds a closed loop comprised of a single-stage of membrane elements and a circulation pump (CP). Permeate is produced at a rate equal to the flowrate of the HP pump. Brine is recirculated without depressurization. When a desired recovery percentage is reached, brine is flushed out of the system, displaced by feedwater from the HP pump. The exchange of brine and feedwater is executed without stopping the HP pump or producing permeate and without depressurizing the system. The process then returns to closed-circuit operation, during which there is no brine reject stream.
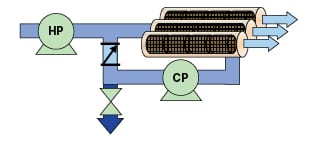
Over 97% recovery has been achieved in closed-circuit RO processes. Energy requirements are reduced because the average membrane feed pressure required to achieve a given recovery rate is lower. The ability to change recovery at the control panel provides substantial flexibility. The frequent flushing of the system by the brine-feedwater exchange also helps suppress fouling and scaling, such that higher recovery rates can be sustained.
In seawater RO, higher pressures are generally required than those used in industrial RO. This makes energy consumption more important and it limits recovery rates. A typical seawater RO process is illustrated in Figure 4. Like in the closed-circuit industrial RO system, the seawater process uses both a high pressure pump and a circulation pump to feed the membrane array. In addition, an energy recovery device (ERD) is used to remove brine and replace it with feedwater while maintaining system pressure. These devices save energy by recovering hydraulic energy from the reject brine and returning it to the low-pressure feed process, thereby reducing the duty of the HP pump.
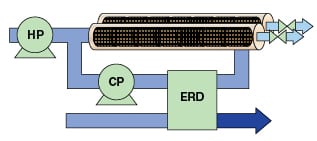
For lower-recovery, higher-pressure applications, such as brine concentration and seawater desalination, an alternative closed-circuit process is used to minimize energy requirements [10,11]. In this process configuration, brine is displaced from the membranes with pressurized feedwater from a side chamber. The exchange, emptying and refilling of the side chamber is done under hydrostatic conditions with almost no loss of pressure energy.
Membranes
RO processes are built around semipermeable membranes capable of filtering out salts. The first membranes were made of cellulose acetate and served as the best available technology until the 1980s, when robust thin-film composite (TFC) membranes were developed [12]. TFC membranes exhibit much higher intrinsic water permeabilities than cellulose acetate membranes because of their extremely thin selective layers. Today, nearly all RO operations use TFC membranes [13]. Despite the great improvements in TFC membrane performance and cost, there are still shortcomings that hinder their application. These limitations include being prone to fouling and being susceptible to attack by oxidizing agents, such as chlorine.
Pumps
The high-pressure pump supplies the pressure needed to push water through the membranes and, in the process illustrated in Figure 1, supplies the crossflow that controls concentration polarization. Typical pressures for industrial water treatment range from 150 to 450 psi (10 to 31 bars). Seawater RO pressures range from 750 to 1,200 psi (52 to 83 bars). Typically, centrifugal-type pumps are used, with sufficient stages and features to meet the pressure requirements. In some high-pressure applications, positive-displacement pumps are preferred because of their generally higher efficiency.
The interstage booster pump shown in Figure 1 adds enough pressure to the concentrate stream to overcome its increased osmotic pressure in later stages. Like the HP pump, the booster pump drives both permeate flow and crossflow. It is sized and operated to “balance” flux and flow between the stages so that all individual membrane elements operate within their design envelopes and are neither over- nor under-utilized. Typical boost pressures for industrial water treatment range from 40 to 200 psi (3 to 14 bars). In some process configurations, a hydraulic turbocharger is used as an inter-stage booster pump, driven by flow and pressure of brine from subsequent stages.
The role of the circulation pump shown in Figures 2 and 3 is to provide crossflow. These pumps only supply enough pressure to overcome friction losses in the flow channels in order to achieve the desired crossflow rate. Typical applied pressures range from 10 to 50 psi (0.7 to 3.4 bars). The circulation pump must be equipped with a shaft seal and bearings designed to handle the incoming pressure of the brine.
Energy recovery devices
Energy recovery devices (ERDs) are installed in the brine stream of seawater RO processes to recover the otherwise wasted hydraulic energy. Initially, these devices were reverse-running pumps or turbines that were mechanically coupled to the HP pump. Net energy-transfer efficiency — the product of turbine efficiency and pump efficiency — could be as high as 80% with very large devices. In the early 2000s, isobaric ERDs were introduced, allowing direct transfer of pressure from the reject stream to the membrane feed stream. These devices offer net transfer efficiencies of 90% or more, and reduce the size and of the HP pump [14]. Turbine ERDs are currently used in some seawater RO applications because of their relatively low cost. ERDs are not typically applied in industrial RO installations because the brine stream does not contain enough energy to justify their cost.
Costs
The cost of water generated by an RO plant comprises capital costs (CAPEX) and operating costs (OPEX). CAPEX per volume of water produced depends upon the construction cost and the amortization rate of the plant, the interest rate (the desired yield on the capital investment) and the plant utilization (load factor). OPEX consists of the fixed costs of staff, insurance and so on, as well as the variable costs of operation and maintenance and repair (including consumables). Energy costs are directly proportional to the price of power and include the pumping energy directly consumed for RO and the energy used for pretreatment, post-treatment and overall plant operations. Additional costs can be incurred for obtaining feedwater and disposing of brine concentrate.
Industrial RO
Assuming typical financial and utilization factors, the CAPEX of an industrial RO unit depends primarily upon the composition of the water being treated and the cost of pre- and post-treatment. However, many industrial RO units can be installed within existing facilities or housed in containers, reducing or eliminating the cost of civil work. The approximate costs for a typical industrial RO unit with a permeate production capacity of 367 gal/min (2,000 m 3/d) at a recovery rate of 75%, assuming a 10-year unit life, is summarized in Table 1. About 70% of the CAPEX indicated is for the equipment, and the balance is for installation. Operation and maintenance (O&M) costs are estimated at $0.57 per 1,000 gal. Brine disposal costs are based on an average U.S. commercial sewage fee of $7.60 per 1,000 gal [17].
Table 1. Approximate Costs of a 367 gal/min (2,000 m3/d) Industrial RO Unit (10-yr life) | ||||||||
Industrial RO | 75% Recovery | 90% Recovery | ||||||
Present value, 10 yr | Cost, gal/d | Cost per 1,000 gal | Percent of total | Present Value, 10 yr |
Cost, gal/d | Percent of total | Cost per 1,000 gal | |
CAPEX | $521,195 | $0.99 | $0.28 | 6% | $521,195 | $0.99 | 12% | $0.28 |
Energy | $480,727 | $0.91 | $0.26 | 6% | $600,909 | $1.14 | 14% | $0.32 |
O&M | $1,148,252 | $2.17 | $0.62 | 13% | $1,148,252 | $2.17 | 26% | $0.62 |
Brine | $6,436,578 | $12.18 | $3.48 | 75% | $2,145,526 | $4.06 | 49% | $1.16 |
Total | $8,586,752 | $16.25 | $4.64 | 100% | $4,415,882 | $8.36 | 100% | $2.39 |
The values in Table 1 clearly indicate that brine disposal is the largest expense item for industrial RO. In this example, increasing recovery from 75% to 90% only slightly increases energy consumption but reduces brine disposal costs by a factor of three, and cuts the overall cost of ownership almost in half.
Seawater RO
Seawater RO installations are typically larger than industrial RO installations, and are tendered for up to 25-year expected lifetimes. A typical CAPEX requirement for a mid-scale seawater RO plant in 2008 was approximately $6 per gallon per day ($1,585 per m 3/d) of permeate output, installed. Therefore, a 10-million gal/d (38,000 m 3/d) plant costs approximately $60 million to build. OPEX is approximately $0.57 per 1,000 gal (about $2.8 million/yr for a 10-million gal/day plant [15]. With a typical energy requirement of 3.2 kWh/m3, including pre- and post-treatment and a power tariff or energy price of $0.10/kWh, the energy cost for a 10 million gal/d plant is $4.4 million per year. The resulting present value costs for 25 years of operation are presented in Table 2.
Table 2. Present Value Costs for a 38,000 m3/d (10 million gal/d) Seawater RO Plant for 25 Years | ||||||||
Seawater RO | Typical energy consumption | 5% Reduced energy consumption | ||||||
Present Value, 25 years | Cost, gal/d | Cost per 1,000 gal | Percent of total | Present Value, 25 years | Cost, gal/d | Cost per 1,000 gal | Percent of total | |
CAPEX | $60,004,542 | $6.00 | $0.66 | 14% | $60,004,542 | $6.00 | $0.66 | 15% |
Energy | $216,953,081 | $21.69 | $2.38 | 52% | $209,569,720 | $20.96 | $2.30 | 51% |
O&M | $138,571,724 | $13.86 | $1.52 | 33% | $138,571,724 | $13.86 | $1.52 | 34% |
Total | $415,529,347 | $41.55 | $4.55 | 100% | $408,145,986 | $40.81 | $4.47 | 100% |
The data in Table 1 indicate that energy comprises more than half the total cost of a 25-year seawater RO operation and more than triple the capital cost of building the plant. Energy-saving methods and technologies can be easily cost-justified. For example, a 5% reduction in seawater RO energy consumption in the 38,000 m 3/d (10 million gal/d) plant considered above would save over $7 million in present value. This value is equivalent to 12% of the cost of ownership of the plant. If a 5% energy savings required a 1% increase in the overall cost of the plant, the investment would be paid back in about three years.
For context, it should be noted that most methods of delivering large quantities of water are expensive. Traditional water distribution and treatment requires building plants and infrastructure. In the developed world, the price to produce 1,000 gal from traditional water supplies ranges from $6 to 15 ($1.52–3.88 per m3) [17]. A comparison of these figures to the costs listed above makes it clear that RO can be a cost-competitive means of water supply.
Potential technology advances
Technology for RO desalination continues to improve. Here are some possible future advances for the key components of RO operations, and their potential value.
Membranes. Ultrahigh-permeability membranes, including graphene membranes or TFC membranes made with carbon nanotubes or aquaporins, have recently received a lot of attention for their potential to reduce the pressure needed to drive permeation, thereby reducing the energy demand of RO [18]. However, high permeability typically reduces salt rejection. Assuming salt rejection can be improved, the amount of energy that can be saved by using ultrahigh-permeability membranes is likely to be very small. Current RO plants already operate near the thermodynamic limit, with the applied pressure being only 10 to 20% higher than the osmotic pressure of the concentrate. Some of this excess pressure is needed to drive crossflow past whatever membrane material is used. Although these membranes might make it possible to use less membrane area and therefore save on capital expense, this would require a redesign of membrane elements because concentration polarization induced by high water fluxes already hinders the performance of current thin-film composite membrane elements. Additionally, membrane fouling is exacerbated at higher water fluxes, further diminishing the value of ultrahigh-permeability membranes for RO.
Reducing or eliminating pretreatment could reduce the cost of RO, but this would require the development of fouling-resistant membranes or membrane coatings. Advances in membrane technology can also reduce the need for the post-treatment currently required for lowering boron and chloride concentrations in permeate to levels suitable for agricultural use. However, membranes with better selective-ion rejection are generally less permeable to water, which increases feed-pressure requirements and energy consumption.
Pumps and ERDs. Although there have been no significant improvements in pump or motor efficiencies specifically for RO applications, pump and plant designers can reduce energy consumption with smart process designs. These include the use of larger centrifugal pumps, with inherently higher efficiencies, and variable frequency drives (VFDs) instead of throttle valves for flow and pressure control. Although VFDs for very large motors can be cost-prohibitive, the HP pump can be fed with a feed booster pump that is equipped with a VFD. A properly controlled booster pump can allow both pumps to operate close to their best efficiency points despite varying process and feedwater conditions. In addition, the use of a feed booster pump allows the use of a HP pump with a higher net positive suction head, which can increase pump efficiency.
Despite the apparently advanced state of isobaric ERDs, innovation continues to broaden the applicability of these devices, improve their performance and reliability, and ultimately reduce the capital and operating costs of seawater RO. ERD efficiencies could be increased by installing additional ERD units, thereby reducing the flowrate to each unit and reducing differential pressure losses, by tightening valve seals and by lowering brine exit velocities [19].
Advanced process designs. The use of multiple stages can lower feed pressure requirements to near the theoretical minimum pressure requirements [20] and potentially allow for more balanced membrane operation. However, the pressure of the concentrate from the final stage is much higher than the feed pressure of the first stage, making it impossible to use isobaric energy-recovery devices in multistage configurations, undermining some of the energy-savings potential of these designs for seawater RO. Also, the additional pumps required add capital costs.
Closed-circuit RO operations provide the energy-saving benefits of multistaging without the need for energy-recovery devices or additional pumps. They facilitate operation at high recovery rates, lowering brine production, the disposal of which is the largest cost component in most industrial RO applications.
Forward osmosis. Forward osmosis uses a water-permeable and salt-rejecting membrane between two solutions of different osmotic pressures. These solutions can be natural resources, waste streams or high-purity solutions. Natural osmosis drives water to permeate through the membrane from the less salty “feed solution” to the more salty “draw solution.” Engineered draw solutions employ specifically selected draw solutes that are separated from the extracted water and recycled to facilitate continuous forward-osmosis operation. However, draw solution regeneration is a cost-intensive step [21]. A recently proposed design is a hybrid system that uses a forward-osmotic contactor to dilute seawater with wastewater effluent prior to RO treatment [22]. However, this system requires two-membrane operation, which adds costs and, for potable water production, the negative public perception about utilizing wastewater effluent as a potable water supply would need to be overcome.
Concluding remarks
Reverse osmosis is a reliable, cost-effective and well-established means of purifying water for domestic and industrial use. RO component and process designs have improved in recent years, lowering water treatment costs. For most industrial RO applications, raising recovery, thereby reducing waste brine generation, represents the greatest cost-saving opportunity. For seawater RO, reducing energy consumption has the greatest prospect for lowering overall costs. Anticipated future technological advances could provide recovery increases and additional energy savings, particularly with closed-circuit RO process designs.
References
1. Service, R.F., Science, vol. 313, p. 1,088, 2006.
2. UNESCO, Water for People, Water for Life, United Nations World Water Development Report, United Nations Educational, Scientific and Cultural Organization, 2003.
3. Schiermeier, Q., Nature, vol. 452, p. 260 2008.
4. National Research Council (U.S.), Committee on Advancing Desalination Technology, “Desalination: A National Perspective,” National Academies Press, 2008.
5. Fritzmann, C., Lowenberg, J., Wintgens, T. and Melin, T., Desalination, vol. 216, p. 1, 2007.
6. Semiat, R., Environ. Sci. Technol., vol. 42, p. 8,193, 2008.
7. Loeb, S. and Sourirajan, S., “Sea Water Demineralization by Means of an Osmotic Membrane,” Advances in Chemistry Series, vol. 38, p. 117, 1962.
8. Efraty, A., U.S. Patent No 7,695,614, 2010b.
9. Efraty, A., Closed Circuit Desalination Series No-4: High Recovery, Low Energy Desalination of Brackish Water by a New Single Stage Method without any loss of Brine Energy, Desalination and Water Treatment, vol. 42, pp. 262–268, 2012.
10. Efraty, A., U.S. Patent No 7,628,921, 2010a.
11. Efraty, A., Barak, R. and Gal, Z., Closed Circuit Desalination — A New Low Energy, High Recovery Technology without Energy Recovery, Desalination and Water Treatment, vol. 31, pp. 95–101, 2011.
12. Larson, R.E., Cadotte, J.E. and Peterson, R.J, Desalination, vol. 38, p. 473, 1981.
13. Lee, K.P., Arnot, T.C., Mattia, D., J. Membr. Sci., vol. 370, p. 1, 2011.
14. Stover, R.L., Desalination vol. 203, p. 168, 2007.
15. Sommariva, C., “Desalination and Water Treatment Economics and Financing,” Balaban Desalination Publications, 2010.
16. Water Desalination Report, Media Analytics, 15 September, [Excerpt], 2008.
17. Global Water Intelligence, vol. 14 (2), September 2013.
18. Elimelech, M. and Phillip, W.A., Science, vol. 333, p. 712, 2011.
19. Stover, R.L. and Andrews, W., IDA Journal vol. 4 (1), p. 38, 2012.
20. Zhu, A.Z., Christofides, P.D. and Cohen, Y., Ind. Eng. Chem. Res., vol. 48, p. 6,010, 2009.
21. McGinnis, R.L. and Elimelech, M., Desalination, vol. 207, p. 370, 2007.
22. Cath, T.Y., Hancock, N.T., Lundin, C.D. Hoppe-Jones, C. and Drewes, J.E., J. Membr. Sci., vol. 362, p. 417, 2010.
Author

Richard Stover is the executive vice president at Desalitech, Inc. (One Gateway Center, Suite 250, Newton, MA 02458; Phone: 1-510-333-2767; Email: [email protected]). Stover has over 27 years of professional experience, specializing in water technologies. He served as chief technical officer and vice president for Energy Recovery, Inc., the leading supplier of energy recovery devices for seawater desalination. He directed the technical/commercial effort that took the company from startup through an IPO. Stover next served as vice president for Oasys Water, developing forward osmosis for brine concentration. Stover’s current work with Desalitech increases the efficiency of industrial, brackish and wastewater treatment with high-recovery RO products. Stover has been granted numerous desalination patents. He was co-recipient of the European Desalination Society’s Sidney Loeb award for outstanding innovation. He has a Ph.D. from the University of California at Berkeley and a B.S. from the University of Texas at Austin, both in chemical engineering.