Improved understanding of crystallizer equipment and operation can aid purification efforts in the CPI
Crystallization is a key purification technique for various sectors of the chemical process industries (CPI). Several approaches for industrial crystallization have evolved over time, and highly specialized crystallizer designs have been developed, especially in long-established industries. Solution crystallization is an important unit operation because the process can generate high-purity products from solutions containing significant levels of impurities with relatively low energy input.
This article is intended to expand knowledge of the various crystallizer configurations, including forced circulation (FC) and draft-tube-baffle (DTB) configurations, which will be discussed in detail.
Classification of crystallizers
Two schemes have been employed to classify crystallization equipment. One uses the method of generating supersaturation, while the other scheme uses the method by which the growing crystals are suspended.
There are six primary methods for generating supersaturation: evaporation, cooling, adiabatic-flash (vacuum) cooling, chemical reaction, antisolvent addition and pH adjustment. However, classification according to the method of generating supersaturation is not entirely satisfactory, since different means of generating supersaturation may be employed within the same type of equipment. When classifying according to the method of suspending the growing crystals, crystallizers fall into one of four basic types:
1) Mixed-suspension, mixed product removal (MSMPR) crystallizers.
Also sometimes called circulating magna crystallizers, this type of equipment circulates the growing crystals through the zone of the crystallizer where the supersaturation conditions are generated. This may be accompanied by mixed- or classified-product removal, and with or without destruction of fines.
2) Circulating liquor/classified- suspension crystallizers.
In this type, only the liquor or a weak slurry is circulated, while the bulk of the growing crystals are not circulated. Supersaturation is imparted to the liquor in one part of the equipment, whereupon this liquor is circulated to another area where it relieves the supersaturation on growing crystals. This type of crystallizer is also available with or without fines destruction capabilities. The units are usually identified as Krystal- or Oslo-type crystallizers.
3) Scraped-surface crystallizers.
Crystallization is induced by indirect heat exchange with a cooling medium at the heat-transfer surface, which is continuously scraped and agitated to minimize fouling. This type of equipment employs vertical tanks with scrapers or horizontal pipes.
4) Tank crystallizers.
Crystallization is produced by cooling the feed solution in either static or agitated tanks by natural convection and radiation, by surface cooling through coils or a jacket, programmed evaporative cooling, reaction or antisolvent methods.
MSMPR crystallizers
The term MSMPR carries the assumption that a perfectly uniform product mixture exists in the mixed slurry of the active volume, where the percentage of suspended solids and crystal size distribution (CSD) are perfectly uniform. This uniformity is also present at the point of product discharge. In addition, it is assumed that the mother liquor has the same residence time as the solids. Thus, there is no deliberate attempt to control the residence time of the crystals of any size.
Forced-circulation crystallizer
The FC crystallizer (Figure 1) is a type of MSMPR unit. This design usually has the lowest capital cost per pound of product generated. The feed usually enters the downpipe at a point following the discharge of the product slurry to the solid-liquid separation device. The combined stream, consisting of fresh feed and recirculated slurry, is pumped via an axial flow pump through the circulating pipe to a vertical or horizontal heat exchanger, where it is heated by condensing steam.
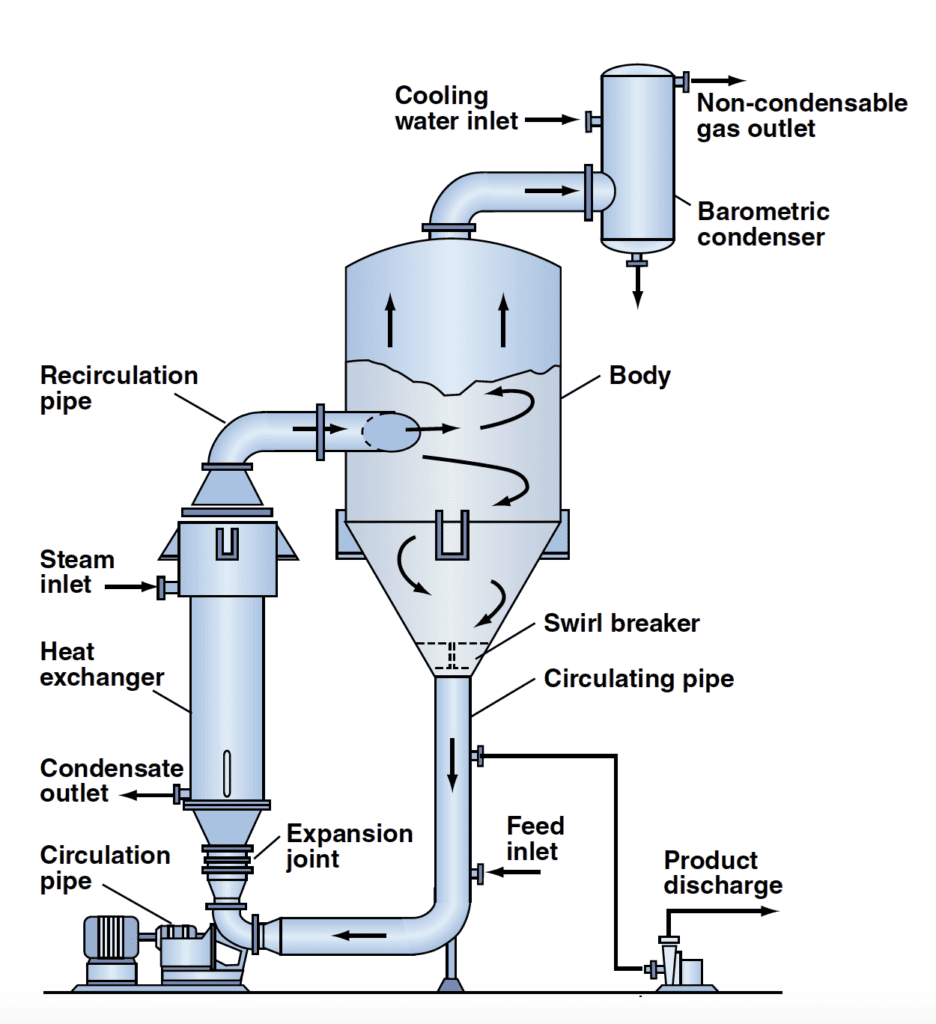
This slurry-and-feed stream is introduced into the crystallizer below the liquid surface in the vapor body, the part of the FC where flashing occurs. It mixes with the slurry at the point of feed entry and raises the local temperature to cause flashing (evaporation under vacuum) at the liquid surface. The flashing causes cooling at the surface, resulting in supersaturation that is relieved as crystal growth occurs or as new nuclei are formed in the active volume. FC units operate with a high circulation rate, which limits scaling. The lowered scaling enables the unit to evaporate solutions that have scaling solutes, such as calcium sulfate.
At times, FC crystallizers can be designed for classified product discharge, usually by suspending an elutriation step beneath the crystallizer body. Introduction of mother liquor to the lower portion of the leg fluidizes the particles prior to discharge, and selectively returns the smaller crystals to the body for future growth. Many NaCl plants have such a process step for both classified product discharge and for keeping a suspension of calcium sulfate in the unit to reduce scaling. The concept is that maintaining small CaSO4 crystals in the vessel encourages growth on the crystal, rather than forming scale throughout the system.
Another modification of an FC unit involves a conical inlet for the slurry to flow into the crystallizer. The conical entrance improves mixing in the body, allowing better dispersion of the supersaturation and improving the uniformity of the slurry at the boiling surface. This installation may also be combined with baffling, in order to allow removal of fines and clear-liquor advance (removing mother liquor without removing crystals). This builds the slurry density beyond its natural level.
An important point is that both classified product removal and fines destruction are not entirely consistent with the MSMPR properties regarding residence, because these practices are deliberate attempts to modify the residence time of the crystals based on their size.
The heat exchanger in an FC unit usually has a one- or two-pass configuration, often with 1.5-in., 12-gauge tubes. It is essential to limit the temperature differential between the steam and the slurry in the heat exchanger to avoid boiling in the tubes, which can lead to scaling, plugging and excessive nucleation. In addition, there must be adequate liquid head at the point of entry to the body (submergence of the slurry leaving the heat exchanger) to avoid flashing in the recirculation line. Doing this avoids premature flashing in the heat exchanger tubes and the inlet piping prior to entry into the crystallizer tank. Another aspect of the FC crystallizer is the use of an axial-flow pump to achieve high slurry flow with reduced crystal breakage and secondary nucleation.
In an adiabatic evaporative-cooling FC unit (where the heat exchanger is omitted), the feed is introduced to the body at a position adequately below the liquid-vapor surface to avoid flashing during the mixing process.
In both configurations, supersaturation is minimized by high circulation rates (typically about 7 ft/s) and by limiting the temperature drop at the liquid-vapor surface to 3–8oF, with some inorganic salt slurries being limited to a 1–5oF decrease.
For some applications, supersaturation is generated by indirect cooling, as opposed to evaporation. In this case, the process flowrate is designed to operate at a low temperature drop (0.5–5oF) and low log-mean-temperature-difference (LMTD) across the heat exchanger. The goal is to stay within the metastable zone to reduce fouling on the tubes. The end result is usually a large surface area, aimed at achieving the required heat transfer and improve on-stream time. The difference between an effective design versus a poor design could be a matter of running for weeks or months versus hours or a few days prior to washout.
Vortexing in the main body of the crystallizer can cause a supersaturated slurry to bypass the boiling surface, resulting in high levels of supersaturation within the body, variable nucleation rates and cycling of the CSD between fine particles and coarse crystals as the crystal surface area varies. A number of design features are used to minimize this problem, including the following:
• Installation of a vortex breaker in the cone of the crystallizer
• Maintaining the slurry inlet nearly tangential to the contour of the crystallizer body, with the angle depending on the vessel diameter
• Keeping sufficient submergence of the feed as it enters the crystallizer body, thereby preventing flashing and vortexing
• Adjusting slurry inlet velocity through the recirculation line to avoid a jet across the crystallizer tank, and unfavorable vortexing. An important parameter here is the Froude number, a dimensionless number used to evaluate the influence of gravity on flow
Froude number can be expressed as:
NFr = V2/(D ⋅ g) (1)
Where V is slurry velocity in ft/s; D is the vessel diameter in feet and g is the gravitational constant (32.2 ft/s2). For example, a light organic slurry with a viscosity of 1–3 cP and a specific gravity of 1.2–1.3 would suggest a NFr value that is less than 0.14.
FC units typically range in diameter from 2–20 ft, with some units being as large as 40 ft. They are especially useful for high evaporation loads. A unit used for evaporating water at 380 mm Hg can typically be designed to handle 250–300 lb/h ft2. The CSD is wide, with a theoretical coefficient of variation (CV) of 50%. In reality, the CV is often in the 20–40% range due to deviations from perfect mixing.
Nucleation and crystal growth
The MSMPR configuration can be utilized to determine the kinetics of nucleation and growth for a system. Both growth and nucleation are dependent on supersaturation, with nucleation often having a higher order of dependence on supersaturation versus growth.
Nucleation can be expressed as:
B0 = kl MTjsb (2)
Where B0 is the number of nuclei formed per unit volume per unit time; kl is the rate constant function of temperature; MT is the slurry density; b and j are power functions; and s is supersaturation concentration.
Higher MT values (slurry densities) promote secondary nucleation, but also lower the level of supersaturation. The goal is to find a balance with operating conditions to maximize size distribution. Other formulas include agitation speed raised to a power. Often b = 1–3 and j = 1.
Crystal growth can be expressed as:
G = k2 sg = dL/dt (3)
Where G is the growth rate of a characteristic crystal face chosen to represent crystal size. Change in characteristic length (k2) is a function of temperature, agitation, impurities and the system; s is supersaturation concentration; g is system-specific.
Combining Equations (2) and (3) yields the following:
B0 = k3 Gi MT j (4)
Where i = b/g. A critical kinetic parameter is the value of i, which determines the relative dependence on supersaturation of nucleation versus growth. The population-density model is used to determine the kinetics. An example of the determination of i is given in Ref. 3.
The traditional operation of an FC crystallizer affords limited flexibility in changing the size distribution.The following equation shows that the ratio of median crystal size (Lm) for two different conditions is:
Lm2/Lm1 = (τ2/τ1)(i-1)/(i+3) (5)
If one changes the residence time (τ), with everything else kept the same, the following is predicted: For i < 1 (which is rare), the median crystal size decreases slightly with an increase in residence time; for i = 1, theoretically, Lm remains the same, and for i > 1, the median particle size would increase.
The change is not great. For example, when i = 2, Lm increases only 15% when the residence time is doubled. This suggests that residence-time changes are not an effective means for increasing particle size.
Figure 2 is a graphical presentation of Equation (5), which demonstrates limited improvement in particle size for i values in the 1–2 range, which is commonly seen. Other than adjusting the residence time or slurry density, the FC crystallizer offers little potential to change the CSD. Changing operating conditions, such as residence time for an MSMPR has a small effect on the mean particle size and CV.
Greater control of the CSD is possible by adjusting the residence time of the crystals in a certain size range, such that it differs from the residence time of the liquid phase. This can be accomplished by fines destruction and classified product removal, which are deviations from a true MSMPR machine. A cautionary note for classified product removal is that these units have a tendency to cycle (even with fines destruction) and while the CV is decreased, the mean particle size may decrease due to the cycling.
Draft-tube-baffle crystallizers
Mechanical impellers and agitators can impact the level of secondary nucleation and breakage. For this reason, low-speed impellers in draft tubes are at times placed in the body to reduce the shear forces seen by the FC circulating pump. The draft tube is an efficient design to suspend solids with lower power input.
Figure 3 depicts a typical draft tube baffle (DTB) crystallizer. In this device, a slow-moving (60–125 rpm) impeller is installed in a draft tube, which circulates the slurry upward to the boiling surface where the supersaturation created by evaporation and cooling is relieved with nucleation and growth.
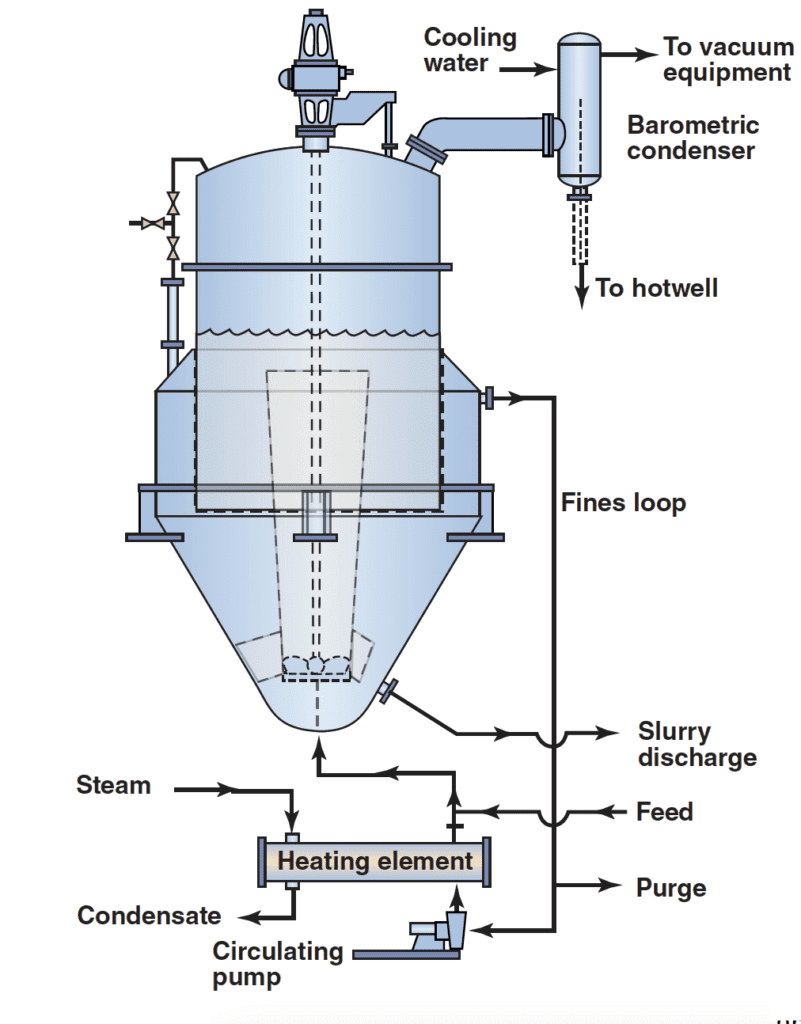
The high flowrates from the agitator are mixed with the feed stream to limit surface cooling to approximately 1–2oF during flashing. This limitation keeps the supersaturation at a low value. Cooled slurry is returned to the bottom of the vessel through the space between the outside of the draft tube and an annular baffle. It then mixes with the heated slurry returning from the heat exchanger or with an adiabatic feed.
The active volume includes volumes inside and outside the draft tube, but not areas behind the baffle. DTBs usually contain slurry equal to 25–50% of apparent settled volume. This active volume is circulated one to four times per minute, which limits nucleation and scaling on the vessel walls while promoting growth on the existing crystal surfaces. Crystal residence times are usually 4–6 h, with the production rates often ranging from 60–85 kg/m3 h. The period between washouts often increases with longer residence time and slurry density as a result of decreased supersaturation and scaling.
The annular baffle area functions as a settling zone through which a stream of mother liquor and fines is separated from the slurry in the active volume. The segmentation is due to differential gravitational settling. The result is a residence time for the fines that is less than the residence time for the product. The CSD in the body is controlled by adjusting the vertical velocity of the mother liquor in the baffle areas and controlling the maximum crystal size that will be removed and dissolved. This can be achieved either by changing the flowrate in the baffle area or the amount of area used for the baffles. The maximum size of the crystals (Lf) that is theoretically removed by the baffle can be used to modify the CSD of the product. The term Lf has a hindered settling velocity equal to the free vertical velocity. If Lf is too large, the increased supersaturation generated by recrystallizing the dissolved fines can cause nucleation in the unit, resulting in periodic upsets in the system and cycling of the CSD.
Increasing the percent solids in the slurry to a concentration greater than the “natural make” (the percent solids without other means of building slurry density) is at times achieved by withdrawing a stream of mother liquor from the upper portion of the baffle zone. This procedure thickens the slurry in the body. This practice is referred to as clear liquor advance (CLA) or double-discharge operation (DDO). It is employed for systems that have low natural make. A larger crystal size may result due to lower levels of supersaturation (more crystal surface area in the slurry), increased residence time for the solids and removal of fines in the CLA. Potential downsides could include increases in secondary nucleation and crystal breakage. High slurry densities tend to reduce the baffle efficiency. To improve performance, a lamella plate is installed in the crystallizer body to direct flow vertically at the baffle entrance, or by the installation of altering doughnut baffles in the settling zone behind the regular baffles. These doughnuts dissipate large liquid eddies that can trap and carry out undesirable larger crystals.
A fines-destruction system produces larger crystals with a narrower size distribution. Ideally, the heat exchanger would supply enough heat to both satisfy the evaporation requirements and to raise the temperature of the low-solids slurry removed from the baffle to destroy unwanted fines. For applications where the only heat removed is that which is required for adiabatic cooling of the feed, fines destruction is achieved by selectively removing a weak slurry stream containing fines and redissolving them via dilution prior to their return to the crystallizer, often in the region below the impeller.
This technique increases supersaturation in the crystallizer body, which increases both crystal growth and nucleation rates. The reason is that excess fines are destroyed by the heat of dilution and then the dissolved solute is recrystallized in the body. The result is a unit that operates at higher internal production rates, due to production of both product crystals and recrystallization of dissolved fines.
The fines-destruction stream, while low in percentage of suspended solids, can often represent a flow that is a multiple of the product underflow stream. Thus, the recrystallization of the dissolved fines can represent a significant percentage of the internal production of the unit. In comparison to the product stream, this configuration deliberately reduces the residence time of the crystals of size Lf or less. Some fines also migrate to the product stream. The ratio of the product crystal residence time to the fines residence time is referred to as R, or τP/τF.
Fines destruction, in the form of accelerated removal and destruction of fine crystals ≤ Lf, can be used to increase the product size. With fines dissolution and recycling to the crystallizer, the supersaturation and production rate increase, thereby increasing the nucleation rate (fines are destroyed) and the growth rate, yielding a larger CSD. Under some conditions, a bimodal distribution with a small peak in the 0 to Lf size range is obtained. A first-order estimate of size improvement is:
This indicates that the size increase is dependent on R, Lf and i. Lm is the median crystal size.
One can then determine the impact of various combinations of Lf and R in order to yield the desired product size. Frequently, Lf is in the 20–80 µm range, and there is a large impact of increasing R, which is often in the 4–10 range. Note that, as expected, the ability to improve the size distribution diminishes as the value of i increases.
Equation (6) indicates that the potential size enlargement is dependent on R, Lf and the relative kinetic order, i. This relationship is shown for a typical inorganic salt in Figure 4. Note that only increasing Lf yields moderate size improvement, with the negative impact of significant production loss due to dissolution of the fines. A more advantageous method is to increase the value of R, and remove the fines at a smaller size. The production loss is decreased while the circulation rate for fines removal is increased. The shadowed area in Figure 4 represents the approximate operating regime for most industrial crystallizers.
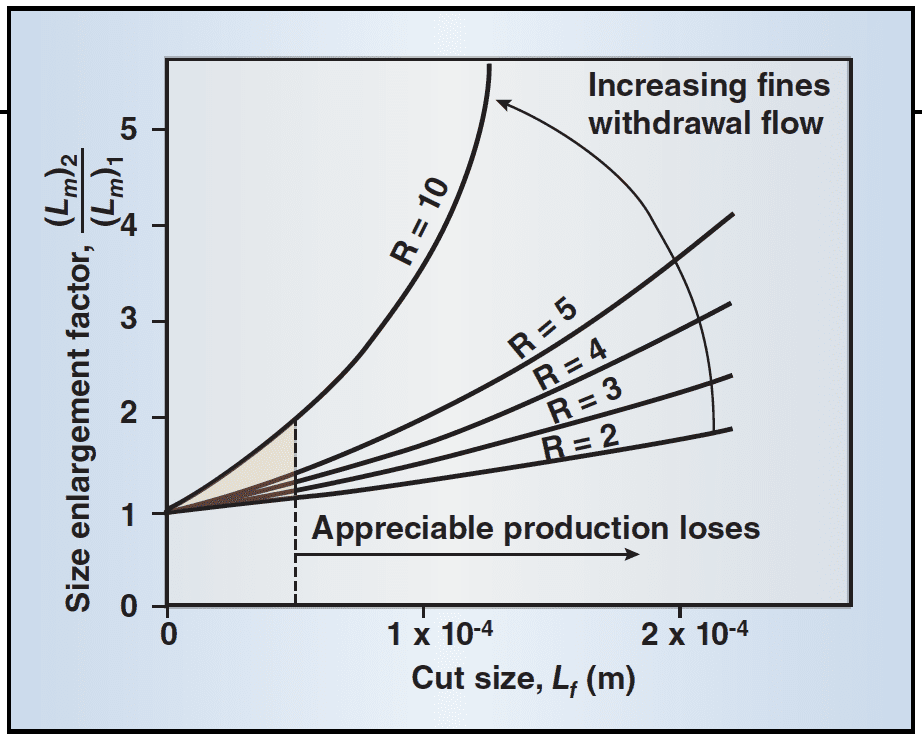
In a sense, a DTB is a hybrid MSMPR, with the active volume resembling the MSMPR mode, but coupled with a classification zone. The DTB has two distinct volumes at play, the crystallization active volume and the baffle volume used as a clarifier. From a kinetic standpoint, the crystallization zone may be referred to as an MSMPR unit. People may initially think that there are no fines in the product stream and that they all move to the baffles. This is not the case; there are fines in the product.
Further CSD improvements can be achieved with an elutriation device. This element will normally be coupled with a fines-destruction system to avoid upsetting the percentage of solids and the dynamics in the unit, which can lead to cycling of the product CSD.
When destruction of fines is not desired, the baffles are eliminated and the internal circulation rate is designed to minimize nucleation in the active volume suspension. This configuration is called a draft tube (DT) mode of operation.
Edited by Scott Jenkins
References
1. Facts at your Fingertips, MSMPR Crystallization. Chem. Eng., October 2010, p. 31.
2. Sutradhar, B., Coping with Crystallization Problems, Chem. Eng., March 2004, pp. 46–52.
3. Schweitzer, P.A., “Handbook of Separation Techniques for Chemical Engineers,” 3rd ed.
McGraw Hill, New York, 1997.
4. “Perry’s Chemical Engineer’s Handbook,” 8th ed., McGraw Hill, New York, 2008.
5. Couper, James. “Chemical Process Equipment: Selection and Design.” Gulf Professional Publishing, Houston, 2010.
Author

Wayne J. Genck is president of the chemical engineering consulting firm Genck International (3 Somonauk Ct., Park Forest, Ill. 60466; Phone: 708-748-7200, Email: [email protected]), specializing in the design and troubleshooting of crystallization and precipitation processes. He has consulted for over 230 industrial clients in the fine, specialty and commodity chemicals, and pharmaceutical sectors. He is a frequent lecturer at in-house seminars and public courses, and wrote the chapter on crystallization for the 8th ed. of Perry’s [4]. Genck holds a Ph.D. in chemical engineering from Iowa State University.